THE ROLE OF PROTEIN CHAPERONES IN THE SURVIVAL FROM ANTHRACYCLINE-INDUCED OXIDATIVE STRESS IN SACCHAROMYCES CEREVISIAE.
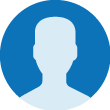
- College of Pharmacy and Pharmaceutical Sciences, Florida A&M University. Tallahassee, FL. USA.
- Departments of Radiation Oncology and Biochemistry, Emory University, Atlanta, GA. USA.
- Abstract
- Keywords
- References
- Cite This Article as
- Corresponding Author
Several S. cerevisiae deletion strains involving heat-shock response factors were among the most sensitive mutants identified in a previous genetic screen for doxorubicin hypersensitivity. These strains included ydj1Δ, ssz1Δ and zuo1Δ mutants. In addition, new1Δ, whose function was unknown, also displayed significant sensitivity to anthracyclines. We further investigated the basis for the sensitivity of these mutants. We determined that heat-shock could partially rescue the sensitivity of the strains to doxorubicin, including the homologous recombination mutant rad52Δ, which is sensitive to doxorubicin-mediated DNA double strand breaks (DSBs). However, none of the heat-shock response mutants were sensitive to DSBs, but were highly sensitive to reactive oxygen species (ROS) generated by quinone-ring-containing agents, such as anthracyclines and menadione. A fluorescent-based assay indicates that doxorubicin causes protein aggregation. Interestingly, the disaggregase mutant hsp104Δ is not sensitive to anthracyclines or menadione suggesting that Hsp104p does not play a role in disaggregating doxorubicin-induced protein aggregates. However New1p, which has been recently shown to be a novel disaggregase, is essential for cell viability after exposure to anthracyclines and menadione and it is not involved in thermotolerance. Our data suggest that in S. cerevisiae, doxorubicin produces protein aggregation through ROS and requires Ydj1p and New1p for resolution.
- Piekarski, M. and A. Jelinska, Anthracyclines still prove effective in anticancer therapy. Mini Rev Med Chem, 2013. 13(5): p. 627-34.
- Minotti, G., et al., Anthracyclines: molecular advances and pharmacologic developments in antitumor activity and cardiotoxicity. Pharmacol Rev, 2004. 56(2): p. 185-229.
- von Minckwitz, G. and M. Martin, Neoadjuvant treatments for triple-negative breast cancer (TNBC). Ann Oncol, 2012. 23 Suppl 6: p. vi35-9.
- Dal Ben, D., et al., DNA topoisomerase II structures and anthracycline activity: insights into ternary complex formation. Curr Pharm Des, 2007. 13(27): p. 2766-80.
- Cummings, J., et al., The enzymology of doxorubicin quinone reduction in tumour tissue. Biochem Pharmacol, 1992. 44(11): p. 2175-83.
- Minotti, G., et al., Doxorubicin cardiotoxicity and the control of iron metabolism: quinone-dependent and independent mechanisms. Methods Enzymol, 2004. 378: p. 340-61.
- Toldo, S., et al., Comparative cardiac toxicity of anthracyclines in vitro and in vivo in the mouse. PLoS One, 2013. 8(3): p. e58421.
- Wallace, K.B., Doxorubicin-induced cardiac mitochondrionopathy. Pharmacol Toxicol, 2003. 93(3): p. 105-15.
- Lenneman, A.J., et al., Heart transplant survival outcomes for adriamycin-dilated cardiomyopathy. Am J Cardiol, 2013. 111(4): p. 609-12.
- Mordente, A., et al., Anthracyclines and mitochondria. Adv Exp Med Biol, 2012. 942: p. 385-419.
- Andre, F. and C.C. Zielinski, Optimal strategies for the treatment of metastatic triple-negative breast cancer with currently approved agents. Ann Oncol, 2012. 23 Suppl 6: p. vi46-51.
- Abdallah, H.M., et al., P-glycoprotein inhibitors of natural origin as potential tumor chemo-sensitizers: A review. J Adv Res, 2015. 6(1): p. 45-62.
- Deng, J., et al., Elevated glutathione is insufficient to protect against doxorubicin-induced nuclear damage in heart in multidrug resistance associated protein 1 (Mrp1/Abcc1) null mice. J Pharmacol Exp Ther, 2015.
- D'Incalci, M., DNA-topoisomerase inhibitors. Curr Opin Oncol, 1993. 5(6): p. 1023-8.
- Xia, L., et al., Identification of genes required for protection from doxorubicin by a genome-wide screen in Saccharomyces cerevisiae. Cancer Res, 2007. 67(23): p. 11411-8.
- Morimoto, R.I., The heat shock response: systems biology of proteotoxic stress in aging and disease. Cold Spring Harb Symp Quant Biol, 2011. 76: p. 91-9.
- Richter, K., M. Haslbeck, and J. Buchner, The heat shock response: life on the verge of death. Mol Cell, 2010. 40(2): p. 253-66.
- Kampinga, H.H. and E.A. Craig, The HSP70 chaperone machinery: J proteins as drivers of functional specificity. Nat Rev Mol Cell Biol, 2010. 11(8): p. 579-92.
- Young, J.C., Mechanisms of the Hsp70 chaperone system. Biochem Cell Biol, 2010. 88(2): p. 291-300.
- Glover, J.R. and R. Lum, Remodeling of protein aggregates by Hsp104. Protein Pept Lett, 2009. 16(6): p. 587-97.
- Lum, R., et al., Evidence for an unfolding/threading mechanism for protein disaggregation by Saccharomyces cerevisiae Hsp104. J Biol Chem, 2004. 279(28): p. 29139-46.
- Nagelkerke, A., et al., The unfolded protein response as a target for cancer therapy. Biochim Biophys Acta, 2014. 1846(2): p. 277-84.
- Jego, G., et al., Targeting heat shock proteins in cancer. Cancer Lett, 2013. 332(2): p. 275-85.
- Mahalingam, D., et al., Targeting HSP90 for cancer therapy. Br J Cancer, 2009. 100(10): p. 1523-9.
- Inoue, Y., et al., Yeast prion protein New1 can break Sup35 amyloid fibrils into fragments in an ATP-dependent manner. Genes Cells, 2011. 16(5): p. 545-56.
- Eisenman, H.C. and E.A. Craig, Activation of pleiotropic drug resistance by the J-protein and Hsp70-related proteins, Zuo1 and Ssz1. Mol Microbiol, 2004. 53(1): p. 335-44.
- Alani, E., R.A. Reenan, and R.D. Kolodner, Interaction between mismatch repair and genetic recombination in Saccharomyces cerevisiae. Genetics, 1994. 137(1): p. 19-39.
- Tishkoff, D.X., et al., A novel mutation avoidance mechanism dependent on S. cerevisiae RAD27 is distinct from DNA mismatch repair. Cell, 1997. 88(2): p. 253-63.
- Tkach, J.M. and J.R. Glover, Nucleocytoplasmic trafficking of the molecular chaperone Hsp104 in unstressed and heat-shocked cells. Traffic, 2008. 9(1): p. 39-56.
- Santoso, A., et al., Molecular basis of a yeast prion species barrier. Cell, 2000. 100(2): p. 277-88.
- Berlett, B.S. and E.R. Stadtman, Protein oxidation in aging, disease, and oxidative stress. J Biol Chem, 1997. 272(33): p. 20313-6.
- Szumiel, I., Ionizing radiation-induced oxidative stress, epigenetic changes and genomic instability: the pivotal role of mitochondria. Int J Radiat Biol, 2015. 91(1): p. 1-12.
- Havaki, S., et al., The role of oxidative DNA damage in radiation induced bystander effect. Cancer Lett, 2015. 356(1): p. 43-51.
- Monneret, C., Recent developments in the field of antitumour anthracyclines. Eur J Med Chem, 2001. 36(6): p. 483-93.
- Langer, S.W., Dexrazoxane for the treatment of chemotherapy-related side effects. Cancer Manag Res, 2014. 6: p. 357-63.
[Jana S. Miles, Samantha J. Sojourner, Lahcen Jaafar, Aurellia Whitmore, Selina Darling-Reed and Hernan Flores-Rozas. (2018); THE ROLE OF PROTEIN CHAPERONES IN THE SURVIVAL FROM ANTHRACYCLINE-INDUCED OXIDATIVE STRESS IN SACCHAROMYCES CEREVISIAE. Int. J. of Adv. Res. 6 (Mar). 144-152] (ISSN 2320-5407). www.journalijar.com
College of Pharmacy and Pharmaceutical Sciences, Florida A&M University